Received: Fri 30, Jun 2023
Accepted: Wed 19, Jul 2023
Abstract
Post-traumatic stress disorder (PTSD) involves dysregulation of stress modulators, particularly corticotrophin-releasing factor (CRF) and glucocorticoid receptor. Endocannabinoid )ECB( signaling usually serves to inhibit the stress response and has been suggested as a potential target for PTSD treatment. The Wnt/β-catenin pathway was found to play a significant role in anxiety and depression. We examined the expression of stress markers in the medial prefrontal cortex (mPFC) and basolateral amygdala (BLA) in a rat model for PTSD, and whether the stress-buffering effects of enhancing ECB signaling are mediated via β-catenin in the mPFC. Rats were exposed to the shock and reminders model for PTSD and injected with the fatty acid amide hydrolase (FAAH) inhibitor URB597 (0.4 mg/kg.). URB597 was found to normalize the dysregulation in stress markers in the mPFC and BLA as well as the anxiogenic phenotype. Importantly, downregulation by viral-mediated gene transfer of β-catenin in the mPFC blocked the stress-buffering effects of URB597 on CRF, a key modulator of the stress response, as well as on CB1r and β-catenin protein levels. We suggest a novel mechanism for the stress-buffering effects of FAAH inhibition on CRF that is dependent on β-catenin activation in the mPFC in a PTSD rat model.
Keywords
Rats, post-traumatic stress disorder (PTSD), cannabinoids, URB597, CB1 receptors, corticotrophin releasing factor (CRF), glucocorticoid receptors (GR), β-catenin
Highlights
Corticotrophin-releasing factor (CRF) is a key modulator of the stress response.
Enhancing endocannabinoids (ECBs) signaling usually inhibits the stress response.
ECBs prevented the development of an anxiogenic phenotype in a PTSD rat model.
PFC β-catenin downregulation blocked the stress-buffering effects of ECBs on CRF.
ECBs’ stress-buffering effects on CRF are PFC-β-catenin dependent in a PTSD model.
1. Introduction
Post-traumatic stress disorder (PTSD) is a complex condition that involves the dysregulation of multiple neurobiological systems, including neuromodulators of stress, such as corticotropin-releasing factor (CRF), CRF1 receptors (CRFr1), and glucocorticoid receptors (GRs). These are dysregulated in psychiatric disorders and mediate anxiety-like behavior and hyperarousal in stressed rats [1-3]. CRF in particular was repeatedly shown to be involved in stress and anxiety regulation and to modulate endocannabinoid (ECB) signaling following stress [4]. The ECB system plays a key role in the modulation of cognitive and emotional responses and is part of the complex circuitry that regulates anxiety and stress [5-11]. The ECB system has been suggested as a therapeutic target for the treatment of severe stress associated with PTSD [12-19]. There is extensive evidence for a bidirectional and functional interaction between glucocorticoids and the ECB system in the stress response [5, 20-22]. Overall, ECBs have been shown repeatedly to modulate the activation and termination of hypothalamic-pituitary-adrenal (HPA)-axis function [23-25]. The ECB components, N-arachidonylethanolamine (anandamide; AEA), 2-arachidonoylglycerol (2- AG), fatty acid amide hydrolase (FAAH), and CB1 receptors (CB1r), are expressed in brain areas modulating stress, fear, emotions, and reward including the basolateral amygdala (BLA) and prefrontal cortex (PFC) [8, 26, 27]. CRF signaling has a stimulatory effect on FAAH enzymatic activity in the amygdala and PFC, which results in reductions in AEA content following stress [4, 28]. Using the exposure to shock and situational reminders (SRs) model of PTSD, we showed that administration of the FAAH inhibitor URB597 (URB) after shock exposure prevented PTSD- and depressive-like behaviors [29-32].
Wnt/β-catenin signaling was found to have a main role in various psychiatric conditions [33-35]. Specifically, β-catenin seems to be implicated in synaptic plasticity, particularly involving emotional learning and memory processes and appears to be involved in disorders associated with strong memory formation such as fear learning in PTSD [36] acting on fear retrieval through the medial PFC (mPFC) [37]. Decreased β-catenin protein levels were found in postmortem human brain samples of suicide victims suffering from major depressive disorder in the mPFC and hippocampus [38-40]. Accordingly, studies have shown that exposure to chronic stress reduces β-catenin in the PFC and hippocampus [41-45] and β-catenin overexpression was found to normalize depression- and anxiety-like behaviors [30, 46-49]. In the BLA, contextual and sensitized fear was accompanied by increased levels of β-catenin [50]. We have recently shown that viral-mediated β-catenin overexpression in the nucleus accumbens (NAc) restored the shock- and reminders-induced increase in anxiety- and depressive-like behaviors, as well as the impaired memory via a CB1r-dependent mechanism [30]. Moreover, when NAc-β-catenin levels were downregulated by viral-mediated gene transfer, the effects of URB597 on anxiety- and depressive-like behaviors and memory were blocked, suggesting that the therapeutic-like effects of FAAH inhibition on behavior are dependent on β-catenin activation in the NAc in a PTSD rat model [30].
CRF, as well as CRFR1 and GRs, are key modulators of physiological, endocrine, and behavioral responses during stress and are bi-directionally associated with the ECB system [5]. In light of this, here we examined the possible involvement of β-catenin in the effects of URB597 on stress neuromodulators abnormalities in fear-related brain circuits. To that end, we downregulated β-catenin in the mPFC by viral-mediated gene transfer and assessed the effects of URB597 on protein and messenger RNA (mRNA) expression of stress markers (CRF, GR, CRFr1) as well as on protein levels of CB1r and β-catenin in the mPFC and BLA. We hypothesized that URB597 prevents the shock- and reminders-induced upregulation in stress markers in the brain and the behavioral anxiogenic phenotype, and that at least some of these restoring effects are mediated via β-catenin in the mPFC.
2. Materials and Methods
2.1. Subjects
Male sprague-dawley rats (60 days old, ~250 g; Invigo Jerusalem, Israel) were caged together according to their group (5 per cage; 59 × 28 × 20 cm) at 22 ± 2°C under 12-hour light/dark cycles (lights turned on at 07:00). Plastic pipes were placed in each cage to enrich the animals’ environment. Rats were allowed water and laboratory rodent chow ad libitum.
2.2. Drug Treatment
The FAAH inhibitor URB597 (0.4 mg/kg; i.p.; Cayman chemicals, MI, USA) was dissolved in 5% dimethylsulfoxide (DMSO), 5% Tween 80 (Sigma, USA) and then diluted with saline (0.9% NaCl) to achieve the final volume. The concentration of DMSO was < 1.5% in the final solution. Control groups were injected with vehicle (DMSO, saline-0.9% NaCl, and tween 80; final DMSO concentration: <1.5%). Drugs were injected one hr after shock exposure. Drug concentration was based on previous studies from our lab [25, 29].
2.3. Shock and Situational Reminders (SRs)
The stress paradigm is based on our previous studies [2, 4, 43]. Rats were exposed to the stressor in a passive avoidance apparatus (50×25×30 cm; manufactured by the University of Haifa workshop), divided into 2 equal-size compartments separated by an automatic guillotine door. On shock exposure day, rats were placed in the light compartment, and after 2 min of exploration, the automatic guillotine door was raised allowing access to the dark compartment. 30 sec after the rat entered the dark compartment, the door was closed and the rat received a 1.5 mA shock for 10 seconds. The no-shock groups received the same treatment with the shock mechanism inactivated.
For SRs, rats were placed in the lighted start chamber for 1 min with the gate closed to prevent them from entering the shock compartment (to avoid extinction). SRs were repeated three times every seven days for a total of 21 days. We used a video camera during the SR days to monitor the rats’ freezing behavior (in sec) during the 60 seconds in the lighted chamber. The percentage of changed pixels between two adjacent 1-s images was calculated, and if the percentage of change in images was <0.05%, the rat was scored as “freezing” [51]. Freezing was defined as the absence of all movement excluding inevitable respiration [52].
For extinction, rats were put back in the light side until they crossed over to the dark side of the shuttle box for 5 days of extinction training. If after 300 seconds the rat did not cross over on its own, the experimenter gently guided it to the dark side. The opening between the two sides was then blocked and no footshock was given. The rat was allowed to freely explore the dark side for 180 seconds, after which it was taken back to the home cage. We measured freezing (sec) in the dark compartment as a measure of conditioned fear.
2.4. Behavioral Tests
All rats were exposed to the same battery of behavioral tests. The tests were carried out in the following order: activity and anxiety-like behavior in a novel open field arena, social interaction test, startle, and extinction. The social test was carried out in the same open field arena as the first test (after 5 min habituation). Tests were separated by a 24-h period and took place between 08:00 a.m. and 1:00 p.m. under dim lighting (15-20 lx).
2.4.1. Open Field (OF)
The open field consists of a closed wooden box (50×50×50 cm). The walls and the floor are painted black and placed under dim red light (<10 lux). The floor is divided by 1-cm-wide white lines into 25 squares measuring 10×10 cm each. The open field arena was thoroughly cleaned between each trial. The movements of the rat were recorded and analyzed for 5 min using a video tracking system (Ethovision ×T 14.0, Noldus Information Technology) to measure anxiety and activity. The anxiety index was calculated as the time spent in the arena center. The activity was measured as the total distance moved (cm).
2.4.2 Social Interaction Test (SIT)
After 5 min habituation in the open field arena (50×50×50 cm), a “partner” rat at the age of 25-28 days was placed in the open field. During the 5-min test, the following social behaviors were scored for duration and frequency: sniffing the partner; physical contact with the partner; climbing over or burrowing under the partner (these are considered pro-social behaviors for analysis); boxing, biting, or threatening the partner (usually these do not occur); self-grooming; and remaining alone-away from the partner (these were analyzed as a-social behaviors). Testing occurred in a dim light (15-20 lx) and was videotaped and analyzed by an experimenter blind to the treatments [53]. A sociality index was calculated for each animal which expresses the percent time that each rat spent engaging in social behavior.
2.4.3 Acoustic Startle Response (ASR)
An acrylic animal holder (8×8×16 cm; Coulbourn Instruments, USA) connected to a piezoelectric accelerometer was placed in a soundproof chamber (25×25×25 cm). Chambers were calibrated for both sensitivity to movement and sound level to assure consistency between chambers and experiments. A high-frequency loudspeaker inside the chamber produced both continuous background noise (68 dB) and acoustic stimuli. Illumination was provided by a white bulb located on the ceiling of the chamber. The animals were placed in the holder and allowed a 5-min acclimatization period with background noise only. After the 5 min habituation, 30 acoustic startle trials (98 dB or 120 dB white noises; 50 ms duration; 20-40 s intertrial interval) were presented over the 68 dB white noise background. The mean startle amplitude was assessed. Mean startle amplitude indicates the average of the response to the 98 and 120 dB in mV [54]. The startle response to both stimulus intensities was averaged as there were no selective effects on one or the other.
2.5. Western Blotting (WB)
Rats were sacrificed and the brains were frozen in liquid nitrogen within 5 min of decapitation and stored at -80°C until dissection. The mPFC and BLA were punched out using a 0.5 mm puncher (coordinates relative to Bregma in mm: mPFC: anteroposterior (AP), +2.9; medial-lateral (ML), ±0.6; ventral (V), -5; BLA: AP, -1.596; ML, ±4.2; V, -8.45; (Figure 1l, 1m) in the results section). Protein levels were determined by the bicinchoninic acid (BCA) protein assay kit (Thermo Fisher Scientific, Waltham, MA, USA) according to the manufacturer's protocol. The samples were then diluted in an SDS sample buffer, boiled (100ºC) for 5 min, and stored at -80ºC. Aliquots were subjected to SDS-PAGE (10% polyacrylamide) and immunoblot analysis.
After 25 µl of protein were loaded, blots were incubated overnight at 4ºC with anti CRF {1:1000; predicted molecular weight (PMW): 21 kDa; Host: Rabbit; abcam (ab184238, GR250583-4), Cambridge,UK}, Anti CRFr1 {1:500; PMW: 51 kDa; Host: Rabbit; abcam (ab229585, Lot GR3220542), Cambridge, UK}, Anti GR {1:100; PMW: 97 kDa; Host: Rabbit; abcam (ab3671, Lot GR3210755-16, GR3210755-17 & GR3210755-18), Cambridge, UK}, Anti CB1r {1:200;PMW: 53kDa; Host: Rabbit; abcam (ab23703, Lot GR3193316 & GR3205675), Cambridge, UK}, or Anti β-Catenin {1:1000; PMW: 86 kDa; Host: Rabbit; abcam (ab32572, Lot GR184212), Cambridge, UK}. This was followed by washing and 1 h incubation with an HRP-linked secondary antibody at room temperature (1: 10,000; goat anti-rabbit IgG; Jackson ImmunoResearch Laboratories, West Grove, PA, USA; 111-035-144). Blots were visualized by enhanced chemiluminescence with ECL (biological industries) and quantified with an XRS charge-coupled device camera (Bio-Rad Laboratories) and Quantity One software. All protein samples were standardized with β-actin (1:1000; PMW: 45 kDa; Host: Rabbit; Cell Signaling (13E5, Lot 5), Danvers, MA, USA) [55]. For antibody specificity, check the supplementary file. The membranes were cropped as different proteins were loaded for each sample at each membrane.
2.6. mRNA Expression Analysis
Rats were euthanized by decapitation and bilateral samples from the mPFC were removed by cryostat using a 0.5 mm puncher. All samples were immediately placed on dry ice and kept at -80°C until further processing. mRNA extraction, cDNA preparation, and quantitative real-time PCR (qRT-PCR) were performed using standard methodology as previously described [56, 57] to detect the expression of mRNAs of the stress markers corticotrophin releasing factor (Crf), corticotropin-releasing-factor-receptor 1 (Crfr1), and nuclear receptor subfamily 3, group C, member 1 (Nr3c1). 1000 ng of total RNA was converted into cDNA using JScript cDNA synthesis Kit (Quanta Biosciences, Gaithersburg, USA). This was followed by real-time SYBR green qRT-PCR amplification using specific primers (Quanta Biosciences, Gaithersburg, USA) according to the manufacturer’s instructions. RT reactions were carried out by a step one real-time PCR system (applied biosystems). Fold-change values were calculated using the ddCt method relative to the housekeeping gene hypoxanthine phosphoribosyl transferase (HPRT). primers (Table S1 in the supplementary information (SI)) were designed and synthesized by Agentek (Tel Aviv, Israel). Primer suitability was determined using standard curve analysis, melting curve analysis, and linearity and threshold [58].
2.7. Viral-Mediated Gene Transfer
The replication-deficient herpes simplex virus (HSV) p1005 vector is a “short-term” vector, derived from herpes simplex virus-1 with a high titer range (3 to 5×108 transduction unit, TU/ml; an illustration of a modified HSV amplicon plasmid is presented in the supplementary file, Figure S1). As previously described [30], all experimental rats were anesthetized via intraperitoneal injection of domitor (2 %, 10 mg/kg, Vetmarket, Modiin, Israel), and after 10 min incubation they were injected with ketamine (10 %, 100 mg/kg, Vetmarket, Israel). The animals were then placed back into the home cage until fully anesthetized. Cranial holes above the mPFC were drilled (Stoelting, USA) relative to bregma (AP = +2.9; ML, ±0.6; DV, -5). After 5 min of rest in the target area (mPFC), 1 μl of an HSV viral vector was injected bilaterally (0.1 μl/min) through a 10-μl hamilton syringe (Hamilton Co., USA) connected to a motorized nanoinjector (Stereotaxic Injector, Stoelting, IL) into the mPFC. An HSV vector was used to downregulate (DRβ) the activity of β-catenin by overexpressing a dominant negative mutant of the protein that lacks its DNA-binding domain [49]. The vector also expresses green fluorescent protein (GFP). A control vector which expresses GFP alone was used as a control. Vectors were injected five days before the shock day; the vectors express their transgenes in vivo within 2-3 hours, with maximal expression from 3-5 days post-injection that lasts only 8 days in vivo. The viral dose was determined by rendering the >90% cell infection rate in brain tissue, diluted in 60% PBS. The needle was held in place for 5 additional minutes before being slowly withdrawn. Animals were allowed 5 days of recovery before behavioral experiments began.
2.8. Perfusion and Immunohistochemistry (IHC)
2.8.1. Perfusion
The paradigm was adopted from an existing protocol with modifications [59]. Experimental rats were anesthetized via IP injection of domitor (0.4 mg/kg). After 10 min incubation, ketamine (60 mg/kg) was injected subcutaneously. Post-fixation brains were kept at -80°C for 24 hours.
2.8-.2. GFP Detection
Brains were sectioned in 35-μm-thick slices using cryostat microtome (LeicBiosystemsms, Deer Park, IL, USA) and stored at 4 °C in PBS. Then, slices were washed three times for 15 min each in 1 × PBS (Sigma-Aldrich, St. Louis, MI, USA). After the washing procedure, the brain slices were mounted on super frost glass slides using PBS as a mounting solution and left to dry for 24 h. Glass slides were then stored at 4°C in a dark chamber. Staining was documented using a confocal microscope at 5×10× and 20× zoom (ZEISS, Jena, Germany).
2.9. Experimental Design
2.9.1. Study Design for Experiment 1 (Scheme 1a)
On day 1, male rats were exposed to a single footshock (1.5 mA, 10 sec) in an inhibitory avoidance apparatus followed by exposure to 3 contextual 1-minute SR of the shock on days 7, 14 and 21. Drugs (Vehicle/ URB597) were injected i.p. 1h after shock exposure. On day 23, rats were sacrificed. In experiment 1, half of the brains were taken to test protein levels of the stress markers (CRF, GR, and CRFr1), CB1, and β-catenin in the relevant brain areas (mPFC and BLA) using WB. The other half of the brains were tested for mRNA expression in the mPFC.
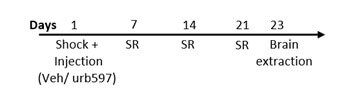
2.9.2. Study Design for Experiment 2 (Scheme 1b)
On day -5 male rats were taken to stereotaxic surgery in which the HSV DRβ vector was injected bilaterally into the mPFC. On day 1, rats were exposed to a single severe footshock (1.5 mA, 10 sec) in an inhibitory avoidance apparatus followed by exposure to contextual 1-minute SR of the shock on days 7, 14, and 21. Drugs (URB597/Veh) were administrated i.p. 1 hr after shock exposure. On days 22-32 rats were exposed to a battery of affective tests: OF, SIT, ASR, and extinction (n=65). On day 39, rats were sacrificed and protein levels of stress markers (CRF, GR, and CRFr1), CB1, and β-catenin were tested using WB in the relevant brain areas (mPFC and BLA).

2.10. Statistical Analysis
The results are expressed as means ± SEM. For statistical analysis, three-way ANOVA, mixed design two-way ANOVA, repeated-measures ANOVA, one-way ANOVA, t-tests, and pearson bivariate correlation tests were used as indicated. All post hoc comparisons were made using tukey's range test. Data were analyzed using SPSS 27 (IBM, Chicago, IL, USA). Homogeneity of variance was confirmed with levene’s test for equality of variances. The normality assumption was examined using the shapiro-wilk test (p<.05).
3. Results
3.1. Experiment 1: The Effects of URB597 on Protein Expression of CRF, GR, CRFr1, CB1, and β-catenin in the mPFC and BLA in Rats Exposed to Shock and Reminders
Rats were exposed to a single severe foot shock followed by SRs. Drugs (URB597 or vehicle) were administered i.p. 1 h after shock exposure. Brains were extracted to measure protein levels of stress markers, CB1, and β-catenin (for detailed study design, Section 2.12 Experimental Design).
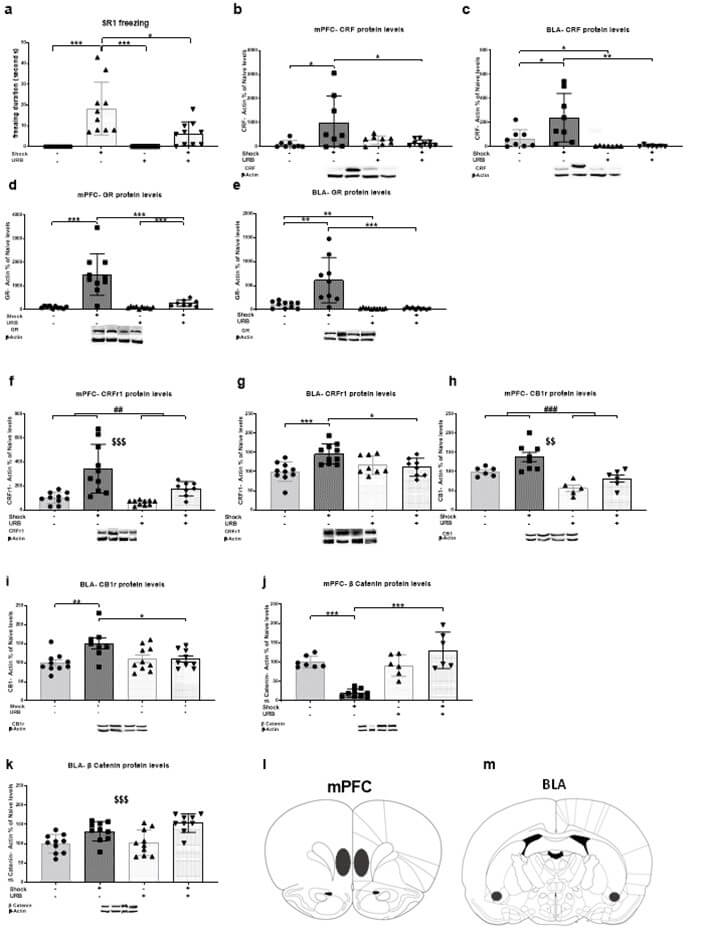
3.1.1. Freezing During the First Situational Reminder
Freezing was measured during the three SRs (each reminder lasted 60 sec) (Figure 1a; n=10 in each group). Two-way ANOVA [shock×drug; 2×2] on the first SR revealed significant effects of shock (F(1,36)=30.91, p<.001), drug (F(1,36)=7.54, p<.01) and drug×shock interaction (F(1,36)=7.54, p<.01). Post-hoc analysis revealed that the shock-vehicle group spent more time freezing compared to all groups (no shock-vehicle and no shock-URB; p<.001; shock-URB: p<.05). This suggests that URB administration in rats exposed to shock and reminders prevented the increase in freezing behavior during the first SR. In addition, the shock-URB group spent more time freezing compared to the no shock-URB group (p<.01). No effects were found on the second and third SRs (data not shown).
3.1.2 CRF
CRF levels were measured in the mPFC (Figure 1d; no shock-vehicle, shock-vehicle, no shock-URB: n=8; shock-URB: n=9) and BLA (Figure 1i; no shock-vehicle, shock-vehicle: n=8; no shock-URB, Shock-URB: n=7). Two-way ANOVA [shock × drug; 2 × 2] revealed significant effects of shock (mPFC: F(1,29)=3.91, p<.05; BLA: F(1,26)=4.70, p<.05), drug (BLA: F(1,26)=12.96, p<.001) and drug×shock interaction (mPFC: F(1,29)=6.73, p<.05; BLA: F(1,26)=4.56, p<.05). Post-hoc analysis revealed a significant increase in CRF protein levels in the shock-vehicle group compared to the no shock-vehicle and shock-URB groups in the mPFC (p<.05) and BLA (no shock-vehicle: p<.05, shock-URB: p<.01). Also, in the BLA the no shock-URB group showed a significant decrease in CRF protein levels compared to the no shock-vehicle group (p<.05). Hence, URB prevented the shock- and reminders- induced upregulation in CRF protein levels in the mPFC and BLA. The same blots were rehybridized with antibodies specific for β-actin to confirm equal protein loading. As there were no differences between the groups in the levels of β-actin in the brain regions we examined, we concluded that the treatment did not affect the levels of β-actin.
3.1.3. GR
GR expression was measured in the mPFC (Figure 1e; no shock-vehicle, shock-vehicle, no shock-URB: n=10; shock-URB: n=8) and BLA (Figure 1j; no shock-vehicle, no shock-URB, shock-URB: n=10; shock-vehicle: n=9). Two-way ANOVA [shock × drug; 2 × 2] revealed significant effects of shock (mPFC: F(1,34)=27.72, p<.001; BLA: F(1,35)=12.25, p<.001), drug (mPFC: F(1,34)=16.78, p<.001; BLA: F(1,35)=20.72, p<.001) and shock×drug interaction (mPFC: F(1,34)=15.93, p<.001; BLA: F(1,35)=12.33, p<.001). Post-hoc analysis revealed a significant increase in GR protein levels in the shock-vehicle group compared to the no shock-vehicle and shock-URB groups in the mPFC (p<.001) and BLA (no shock-vehicle: p<.01; shock-URB: p<.001). Hence, URB prevented the shock- and reminders- induced upregulation in GR protein levels in the mPFC and BLA. Also, in the mPFC, the shock-URB group showed a significant increase in GR protein expression compared to the no shock-URB group (p<.001); in the BLA the no shock-URB group showed a significant decrease in GR protein levels compared to the no shock-vehicle group (p<.01).
3.1.4. CRFr1
CRFr1 expression was measured in the mPFC (Figure 1f; no shock-vehicle, shock-vehicle, no shock-URB: n=10; shock-URB: n=8) and BLA (Figure 1k; no shock-vehicle, shock-vehicle: n=10; no shock-URB, shock-URB: n=8). Two-way ANOVA [shock × drug; 2 × 2] revealed significant effects of shock (mPFC: F(1,34)=24.59, p<.001; BLA: F(1,32)=6.04, p<.05), drug (mPFC: F(1,34)=8.03, p<.01) and shock×drug interaction (BLA: F(1,32)=9.60, p<.01). In the mPFC , a significant increase in CRFr1 protein levels was observed in shocked rats compared to the non-shocked rats and a significant decrease in rats treated with URB compared to vehicle. In the BLA, post hoc analysis revealed a significant increase in CRFr1 protein levels in the shock-vehicle group compared to the no shock-vehicle (p<.001) and shock-URB (p<.05) groups. This suggests that URB597 prevented the shock- and reminders- induced upregulation in CRFr1 protein levels in the PFC and BLA.
3.1.5. CB1r
CB1r expression was measured in the mPFC (Figure 1g; no shock-vehicle, shock-URB: n=6; shock-vehicle: n= 8; no shock-URB: n=5) and BLA (Figure 1l; noshock-vehicle, no shock-URB: n=10; shock-vehicle: n=8; shock-URB: n=9). Two-way ANOVA [shock × drug; 2 × 2] revealed significant effects of shock (mPFC: F(1,21)=9.89, p<.01; BLA: F(1,33)=6.33, p<.05), drug (PFC: F(1,21)=24.41, p<.001) and shock×drug interaction (BLA: F(1,33)=6.54, p<.05). In the mPFC, a significant increase in CB1r protein levels were observed in shocked rats compared to the non-shocked rats and a significant decrease in rats treated with URB compared to vehicle. In the BLA, post hoc analysis demonstrated a significant increase in CB1r protein levels in the shock-vehicle group compared to the no shock-vehicle (p<.01) and shock-URB (p<.05) groups. Thus, URB prevented the shock- and reminders- induced upregulation in CB1r protein levels in the PFC and BLA.
3.1.6. β-catenin
β-catenin expression was measured in the mPFC (Figure 1h; no shock-vehicle, shock-URB: n=7; shock-vehicle: n=9; Noshock-URB: n=6) and BLA (Figure 1m; no shock-vehicle, shock-vehicle, no shock-URB: n=10; shock-URB: n=9). Two-way ANOVA [shock × drug; 2 × 2] revealed significant effects of shock (BLA: F(1,35)=23.20, p<.001), drug (mPFC: F(1,24)=24.55, p<.001), and shock×drug interaction (mPFC: F(1,24)=33.72, p<.001). In the mPFC, post- hoc analysis revealed a significant decrease in β-catenin protein levels in the shock-vehicle group compared to the no shock-vehicle and shock-URB groups (p<.001). Hence, URB prevented the shock- and reminders- induced downregulation in β-catenin protein levels in the mPFC. In the BLA, shocked rats showed increased β-catenin protein levels compared to the non-shocked rats (p<.001).
To summarize, URB administration prevented the shock- and reminders-induced increase in freezing behavior during the first SR. In the mPFC, URB prevented the shock- and reminders-induced upregulation in CRF, GR, CRFr1, and CB1r protein levels and downregulation in β-catenin protein levels. In the BLA, URB prevented the shock- and reminders-induced upregulation in the CRF, GR, CRFr1, and CB1r protein levels.
3.1.7. Correlations between Protein Levels and Behavior
Pearson bivariate correlation tests (Table 1) were conducted between the expression of the different proteins in the brain’s fear circuit and behavior, to examine the association between the freezing levels of the rats during exposure to the first reminder and the expression of CRF, GR, CRFr1, CB1, and β-catenin protein levels.
TABLE 1: Pearson correlations coefficients of freezing levels
during the first situational reminder and protein levels.
Pearson Correlation |
Freezing measure during
SR1 (N) |
|
CRF |
mPFC |
0.801*** (33) |
BLA |
0.624*** (31) |
|
GR |
mPFC |
0.355* (37) |
BLA |
0.324* (38) |
|
CRFr1 |
mPFC |
0.423** (37) |
BLA |
0.367* (38) |
|
CB1
|
mPFC |
0.353 (24) |
BLA |
0.236 (36) |
|
β-catenin |
mPFC |
-0.144 (27) |
BLA |
0.487** (38) |
BLA:
basolateral amygdala; CB1: cannabinoid receptor 1; CRF:
corticotrophin-releasing factor; CRFr1: corticotrophin-releasing factor
receptor 1; GR: glucocorticoids; mPFC: medial prefrontal cortex; N: number of
rats in each group; SR: situational reminder.
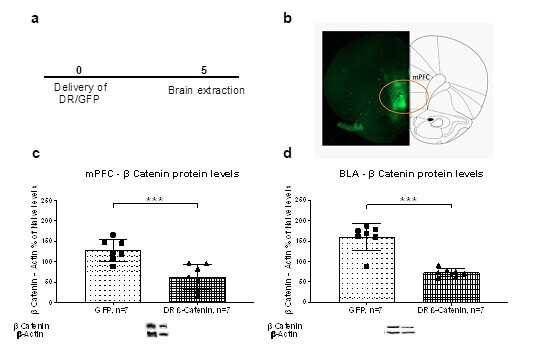
The most notable correlations were found between freezing measures during SR1 and CRF protein levels in the mPFC (r=.801, p<.001) and BLA (r=.624, p<.001), suggesting that increased freezing was associated with increased CRF levels in these brain regions. Other significant correlations were found between freezing and GR levels in the mPFC (r=.355, p<.05) and BLA (r=.324; p<.05); CRFr1 levels in the mPFC (r=.423, p<.01) and BLA (r=.367, p<.05), and β-catenin levels in the BLA (r=.487, p<.01). This suggests that increased freezing was also associated with increased GR and CRFr1 levels in the mPFC and BLA, and increased β-catenin in the BLA.
3.2. Experiment 2: The Effects of Downregulating β-catenin in the mPFC on Behavioral Effects of Shock and Reminders and the Protein Expression of CRF, GR, CRFr1, CB1r, and β-catenin in the mPFC and BLA
We found pronounced effects of shock exposure on protein expression that were mostly normalized by URB. However, we found no effects of URB of normalizing shock-induced changes in gene expression of the stress markers (Figure S1b in the SI). Hence, in the next experiment which aimed to study the mechanisms underlying the buffering effects of URB on stress markers (CB1r) and β-catenin expression, we examined alterations in protein expression. Specifically, we aimed to examine whether the stress-buffering effects of URB are mediated by the Wnt/β-catenin pathway. To that end, we downregulated β-catenin levels in the mPFC of rats exposed to shock and reminders and treated with URB. As shock exposure downregulated the expression of β-catenin in the mPFC, we decided to target the mPFC with a viral vector that downregulates β-catenin.
3.2.1. Verifying β-Catenin Downregulation
In a preliminary experiment, we delivered DR β-catenin vectors into the mPFC (Figure 2a). In one set of rats (n = 14) we measured β-catenin expression in the mPFC and BLA using WB. An independent sample t-test revealed that downregulating β-catenin in the mPFC resulted in a significant downregulation of β-catenin levels in the mPFC [t(12)=4.23, p<.001; Figure 2c] and in the BLA [t(12)=6.59, p<.001; Figure 2d] compared to the GFP group. In a second set of rats (n =6) we verified the accuracy of the injection in the mPFC using GFP detection (Figure 2b).
Next, we examined whether viral-mediated DR of β-catenin in the mPFC can block the therapeutic effects of URB on shock-induced effects on behavior and protein expression, compared to rats injected with GFP (n=8 in each group; Section 2.12. Experimental Design).
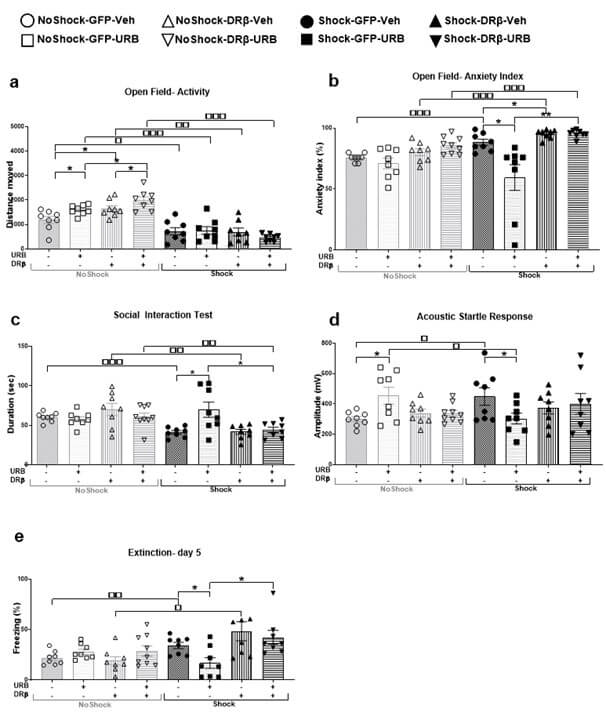
3.2.2. Activity and Anxiety Index in the Open Field (OF) Test
For activity index in the OF test (Figure 3a), mixed design three-way ANOVA [shock×virus×drug; 2×2×2] revealed significant effects of shock (F(1,57)=93.45, p<.001), virus×shock (F(1,57)=8.94, p<.01) and shock×drug (F(1,57)=8.58, p<.01) interactions. Post-hoc analysis revealed a significant decrease in the distance moved in all the shocked groups (shock-GFP-Veh, shock-GFP-URB, shock-DRβ-Veh, shock-DRβ-URB) compared to the non-shocked groups (no shock-GFP-Veh: p<.05; no shock-GFP-URB: p<.001; no shock-DRβ-Veh: p<.01; no shock-DRβ-URB: p<.001), respectively. Hence, exposure to shock and reminders decreased activity in the OF test. Also, the no shock-DRβ-URB and no shock-GFP-Veh groups demonstrated a significant increase and decrease, respectively, in the distance moved compared to the no shock-DRβ-Veh and no shock-GFP-URB groups (p<.05).
For anxiety index in the OF test (Figure 3b), mixed design three-way ANOVA [shock×virus×drug; 2×2×2] revealed significant effects of shock (F(1,57)=7.85, p<.01), virus (F(1,57)=18.64, p<.001), drug (F(1,57)=8.97, p<.01), virus×shock (F(1,57)=7.19, p<.01), virus×drug (F(1,57)=6.11, p<.05) and shock×virus×drug (F(1,57)=5.21, p<.05) interactions. Post-hoc analysis revealed that the shocked groups (shock-GFP-Veh, shock-DRβ-Veh, shock-DRβ-URB) demonstrated a significant increase in the anxiety index compared to the non-shocked groups (no shock-GFP-Veh, no shock-DRβ-Veh, and no shock-DRβ-URB, p<.001), suggesting that exposure to shock and reminders increased anxiety levels in the OF test.
However, the shock-GFP-URB group demonstrated a significant decrease in the anxiety index compared to the shock-GFP-Veh (p<.05) and shock-DRβ-URB (p<.01) groups, suggesting that URB normalized the shock- and reminders-induced increase in the anxiety index, and that downregulating β-catenin in the mPFC blocked the therapeutic-like effect of URB. Also, the shock DRβ-Veh demonstrated a significant increase in the anxiety index compared to the shock GFP-Veh group (p<.05).
3.2.3. Social Interaction Test (SIT)
For SIT (Figure 3c), mixed design three-way ANOVA [shock×virus×drug; 2×2×2] revealed significant effects of shock (F(1,57)=12.75, p<.001), virus×shock (F(1,57)=6.73, p<.05), virus×drug (F(1,57)=5.00, p<.05), and shock×drug (F(1,57)=8.44, p<.01) interactions. Post-hoc analysis revealed that the shocked groups (shock-GFP-Veh, shock-DRβ-Veh, shock- DRβ-URB) demonstrated a significant decrease in social behavior compared to the non-shocked groups (no shock-GFP-Veh: p<.001, no shock-DRβ-Veh: p<.01, no shock-DRβ-URB: p<.01, respectively), suggesting that exposure to shock and reminders decreased social behavior measured in the SIT. However, the shock-GFP-URB group demonstrated a significant increase in social behavior compared to the shock-GFP-Veh and shock-DRβ-URB groups (p<.05), suggesting that URB normalized the shock- and reminders-induced decrease in social behavior, and that downregulating β-catenin in the mPFC blocked the therapeutic-like effect of URB.
3.2.4. Acoustic Startle Response (ASR)
For the ASR test (Figure 3d), mixed design three-way ANOVA [shock×virus×drug; 2×2×2] revealed significant effects of shock×drug (F(1,57)=4.89, p<.05) and virus×shock×drug (F(1,57)=7.17, p<.01) interactions. Post-hoc analysis revealed that the shock-GFP-Veh group demonstrated a significant increase in ASR compared to the no shock-GFP-Veh group (p<.05), suggesting that exposure to shock and reminders increased startle response. However, the shock-GFP-URB group demonstrated a significant decrease in acoustic startle response compared to the shock-GFP-Veh and no shock-GFP-URB groups (p<.05), suggesting that URB normalized the shock- and reminders-induced increase in acoustic startle response. Also, the no shock-GFP-URB group showed d significant increase in startle response compared to the no shock-GFP-Veh group (p<.05).
3.2.5. Extinction Test- Freezing
For the extinction test (Figure 3e), mixed design three-way ANOVA [shock×virus×drug; 2×2×2) revealed significant effects of shock (F(1,57)= 8.43, p<.01), virus (F(1,57)= 6.21, p<.05), shock×drug (F(1,57)= 6.80, p<.05) and shock×virus (F(1,57)= 7.32, p<.01) interactions. Post-hoc analysis revealed that the shocked groups (shock-GFP-Veh and shock-DRβ-Veh) demonstrated a significant increase in freezing behavior during extinction day 5 compared to the non-shocked groups (no shock-GFP-Veh: p<.01 and no shock- DRβ-Veh: p<.05, respectively), suggesting that exposure to shock and reminders increased freezing behavior measured in the extinction test. However, the shock-GFP-URB group demonstrated a significant decrease in freezing behavior compared to the shock-GFP-Veh and shock-DRβ-URB groups (p<.05), suggesting that URB normalized the shock- and reminders-induced increase in freezing, and that downregulating β-catenin in the mPFC blocked the therapeutic-like effect of URB.
3.2.6. CRF
3.2.6.1. PFC
CRF expression was measured in the mPFC (Figure 4a; n=8-9). A mixed design three-way ANOVA [shock×virus×drug; 2×2×2] revealed significant effects of shock (F(1,56)=112.14, p<.001), virus (F(1,56)=14.30, p<.001), drug (F(1,56)=51.55, p<.001), shock×drug (F(1,56)=10.42, p<.01), virus×shock (F(1,56)=39.77, p<.001), virus×drug (F(1,56)=4.99, p<.05) and shock×virus×drug (F(1,56)=9.00, p<.01). Post-hoc analysis revealed that the stressed groups (shock-GFP-Veh, shock-DRβ-Veh, shock-DRβ-URB) demonstrated significant upregulation in CRF protein levels compared to the non-shocked groups (no shock- GFP-Veh, no shock-DRβ-Veh, and no shock-DRβ-URB, p<.001, respectively), suggesting that exposure to shock and reminders upregulated CRF protein expression.
However, the shock-GFP-URB group demonstrated a significant downregulation in CRF compared to the no shock-GFP-URB (p<.05), shock-GFP-Veh (p<.001) and shock-DRβ-URB (p<.001) groups, suggesting that URB normalized the shock- and reminders-induced upregulation in CRF expression and that downregulating β-catenin in the mPFC blocked the therapeutic-like effect of URB. Also, the shock-DRβ-Veh group demonstrated significant upregulation in CRF expression compared to the shock-GFP-Veh group (p<.05). In addition, non-stressed URB-treated rats (no shock-GFP- URB and no shock-DRβ-URB) demonstrated a significant downregulation in CRF expression compared to the non-stressed Veh-treated groups (no shock-GFP-Veh: p<.05 and no shock-DRβ-Veh: p<.01, respectively), indicating that URB downregulated CRF expression in non-stressed rats. Finally, the no shock-DRβ-URB group demonstrated significant downregulation in CRF protein levels compared to the no shock-GFP-URB group (p<.01).
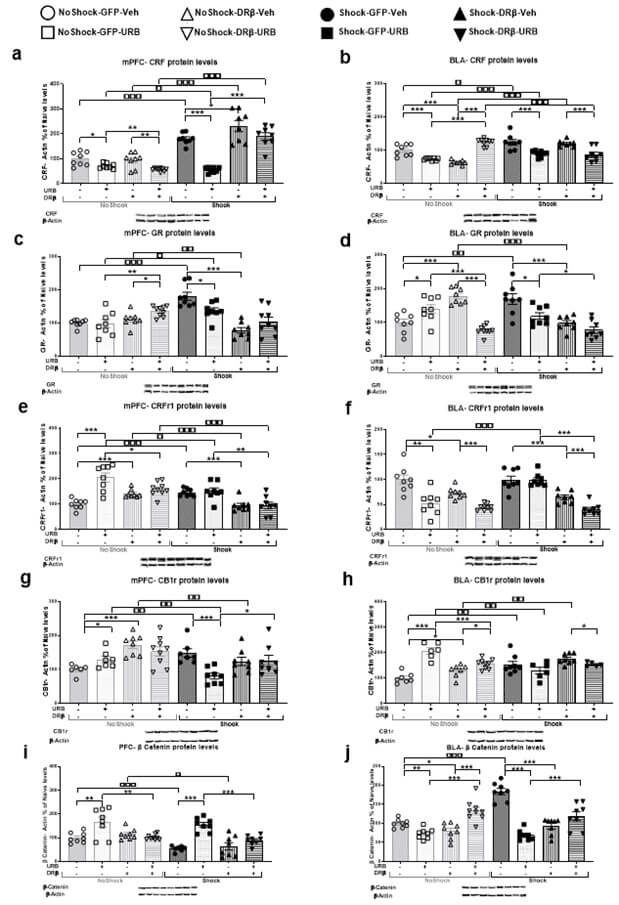
3.2.6.2. BLA
CRF expression was measured in the BLA (Figure 4b; n=8-9). A mixed design three-way ANOVA [shock×virus×drug; 2×2×2] revealed significant effects of shock (F(1,57)=18.46, p<.001), drug (F(1,57)=6.39, p<.05), shock×drug (F(1,57)=52.50, p<.001), virus×drug (F(1,57)=40.36, p<.001) and shock×virus×drug (F(1,57)=37.80, p<.001) interactions. Post-hoc analysis revealed that the stressed groups (shock-GFP-Veh, shock-GFP-URB, and shock- DRβ-Veh) demonstrated a significant upregulation in CRF protein levels compared to the non-shocked groups (no shock-GFP-Veh: p<.05, no shock-GFP-URB: p<.001 and no shock- DRβ-Veh: p<.001, respectively), suggesting that exposure to shock and reminders upregulated CRF protein expression.
However, the shock-GFP-URB and shock-DRβ-URB groups demonstrated a significant downregulation in CRF expression compared to the shock-GFP-Veh and shock-DRβ-Veh groups (p<.001), respectively, suggesting that URB normalized the shock- and reminders-induced upregulation in CRF, and that downregulating β-catenin in the mPFC did not block the therapeutic-like effect of URB. Also, the shock-DRβ-URB group demonstrated a significant downregulation in CRF compared with the no shock-DRβ-URB group (p<.001). Finally, the no shock-GFP-Veh group demonstrated a significant upregulation in CRF protein levels compared to the no shock-GFP-URB and no shock-DRβ-Veh groups (p<.001) and the no shock-DRβ-URB group demonstrated significant upregulation in CRF levels compared to the no shock-DRβ-Veh and no shock-GFP-URB groups (p<.001).
3.2.7. GR
3.2.7.1. PFC
GR expression was measured in the mPFC (Figure 4c; n=8-9). A mixed design three-way ANOVA [shock × virus × drug; 2×2×2] revealed significant effects of virus (F(1,57)=8.76, p<.01), shock (F(1,57)=5.02, p<.05), virus × shock (F(1,57)=42.58, p<.001) and virus × drug (F(1,57)=14.27, p<.001) interactions. Post-hoc analysis revealed that the stressed groups (shock-GFP-Veh and shock-GFP-URB) demonstrated a significant upregulation in GR protein levels compared to the non-shocked groups (no shock-GFP-Veh: p<.001 and no shock-GFP-URB: p<.05, respectively), suggesting that exposure to shock and reminders upregulated GR protein expression. The shock-GFP-URB group demonstrated a significant downregulation in GR compared to the shock-GFP-Veh group (p<.05), suggesting that URB normalized the shock- and reminders-induced upregulation in GR. Nonetheless, the shock-DRβ-Veh group demonstrated significant downregulation in GR expression compared to the shock-GFP-Veh (p<.001) and no shock-DRβ-Veh (p<.01) groups. Also, the no shock-DRβ-URB group demonstrated significant upregulation in GR expression compared to the no shock-DRβ-Veh (p<.05) and no shock-GFP-URB (p<.01) groups.
3.2.7.2. BLA
GR expression was measured in the BLA (Figure 4d; n=8-9). A mixed design three- way ANOVA [shock×virus×drug; 2×2×2] revealed significant effects of virus (F(1,55)=9.98, p<.01), drug (F(1,55)=18.80, p<.001), virus×shock (F(1,55)=18.04, p<.001), virus×drug (F(1,55)=13.96, p<.001) and shock×virus×drug (F(1,55)=36.73, p<.001). Post-hoc analysis revealed that the shock-GFP-Veh group demonstrated a significant upregulation in GR protein levels compared to the no shock-GFP-Veh group (p<.01). The shock-GFP-URB group demonstrated a significant downregulation in GR compared to the shock-GFP-Veh group (p<.05), suggesting that URB normalized the shock- and reminders-induced upregulation in GR. Nonetheless, the shock-DRβ-Veh and shock-DRβ-URB groups demonstrated significant downregulation in GR expression compared to the shock-GFP-Veh (p<.001) and shock-GFP-URB (p<.05) groups, respectively. Also, the no shock-DRβ-Veh group demonstrated a significant upregulation in GR compared to the no shock-DRβ-URB, no shock-GFP-Veh, and shock-DRβ-Veh groups (p<.001). Finally, the no shock-GFP-URB group demonstrated a significant upregulation in GR expression compared to the no shock-GFP-Veh (p<.05) and no shock-DRβ-URB (p<.001) groups.
3.2.8. CRFr1
3.2.8.1. PFC
CRFr1 expression was measured in the mPFC (Figure 4e; n=8-9). A mixed design three-way ANOVA [shock×virus×drug; 2×2×2] revealed significant effects of shock (F(1,56)=15.43, p<.001), virus (F(1,56)=14.07, p<.001), drug (F(1,56)=16.74, p<.001), shock×drug (F(1,56)=14.09, p<.001(, virus×shock (F(1,56)=10.9, p<.001), virus×drug (F(1,56)=9.09, p<.01) and shock×virus×drug (F(1,56)=6.97, p<.01). Post-hoc analysis revealed that the shock-GFP-Veh group demonstrated a significant upregulation in CRFr1 expression compared to the no shock-GFP-Veh group (p<.001), but the other stressed groups (shock-GFP-URB, shock- DRβ-Veh and shock-DRβ-URB) demonstrated a significant downregulation in CRFr1 protein levels compared to the non-stressed groups (no shock-GFP-URB: p<.05, no shock- DRβ-Veh: p<.001 and no shock-DRβ-URB: p<.001, respectively).
However, the shock-DRβ-Veh and shock-DRβ-URB groups demonstrated a significant downregulation in CRFr1 protein levels compared to the shock-GFP-Veh (p<.001) and shock-GFP-URB (p<.01) groups, respectively, suggesting that downregulating β-catenin downregulated CRFr1 protein levels in rats exposed to shock and reminders. Also, The no shock-GFP-URB group showed significant upregulation in CRFr1 protein levels compared to the no shock-GFP-Veh (p<.001) and no shock-DRβ-URB (p<.05) groups. Finally, the no shock-DRβ-Veh group demonstrated significant upregulation in CRFr1 protein levels compared to the no shock-GFP-Veh group (p<.001).
3.2.8.2. BLA
CRFr1 expression was measured in the BLA (Figure 4f; n=8-9). A mixed design three-way ANOVA [shock×virus×drug; 2×2×2)] revealed significant effects of shock (F(1,56)=3.93, p<.05), virus (F(1,56)=57.51, p<.001), drug (F(1,56)=36.01, p<.001), shock×drug (F(1,56)=9.42, p<.01), virus×shock (F(1,56)=11.91, p<.001) and shock×virus×drug (F(1,56)=6.63, p<.05) interactions. Post-hoc analysis revealed that the Shock-GFP-URB group demonstrated a significant downregulation in CRFr1 expression compared to the no shock- GFP-URB group (p<.001). In addition, the shock-DRβ-Veh and shock-DRβ-URB groups demonstrated a significant downregulation in CRFr1 protein levels compared to the shock- GFP-Veh and shock-GFP-URB groups (p<.001) respectively, suggesting that downregulating β-catenin downregulated CRFr1 protein levels in rats exposed to shock and reminders. The shock-DRβ-URB group demonstrated a significant downregulation in CRFr1 expression compared to the shock-DRβ-Veh group (p<.001).
In addition, non-stressed URB-treated rats (no shock-GFP-URB and no shock- DRβ-URB) demonstrated a significant downregulation in CRFr1 expression compared to non-treated groups (no shock-GFP-Veh: p<.01 and no shock-DRβ-Veh: p<.001, respectively), indicating that URB decreased CRFr1 expression in non-stressed rats. Finally, the no shock-DRβ-Veh group demonstrated a significant downregulation in CRFr1 compared to the no shock-GFP-Veh group (p<.05).
3.2.9. CB1r
3.2.9.1. PFC
CB1r expression was measured in the mPFC (Figure 4g; n=6-9). A mixed design three-way ANOVA [shock×virus×drug; 2×2×2)] revealed significant effects of shock (F(1,53)=4.98, p<.05), virus (F(1,53)=11.50, p<.001), shock×drug (F(1,53)=4.84, p<.05), virus×shock (F(1,53)=4.89, p<.05) and shock×virus×drug (F(1,53)=11.93, p<.001). Post-hoc analysis revealed that the shock-GFP-Veh group demonstrated a significant upregulation in CB1r protein levels compared to the no shock-GFP-Veh group (p<.01). Nonetheless, the other stressed groups (shock-GFP-URB and shock-DRβ-Veh) demonstrated a significant downregulation in CB1r expression compared to the non-stressed groups (no shock-GFP-URB and no shock- DRβ-Veh, p<.01, respectively).
However, the shock-GFP-URB group demonstrated a significant downregulation in CB1r compared to the shock-GFP-Veh (p<.001) and shock-DRβ-URB (p<.05) groups, suggesting that URB normalized the shock- and reminders-induced upregulation in CB1r, and that downregulating β-catenin in the mPFC blocked the therapeutic-like effect of URB. Also, the no shock-GFP-Veh group demonstrated a significant downregulation in CB1r protein levels compared to the no shock-GFP-URB (p<.05) and no shock-DRβ-Veh (p<.001) groups.
3.2.9.2. BLA
CB1r expression was measured in the BLA (Figure 4h; n=6-9). A mixed design three-way ANOVA [shock×virus×drug; 2×2×2] revealed significant effects of the drug (F(1,48)=9.91, p<.01), shock × drug (F(1,48)=41.31, p<.001), virus×shock (F(1,48)=6.21, p<.05), virus×drug (F(1,48)=8.11, p<.01) and shock×virus×drug (F(1,48)=8.73, p<.01). Post-hoc analysis revealed that the stressed groups (shock-GFP-Veh and shock-DRβ-Veh) demonstrated a significant upregulation in CB1r protein levels compared to the non-shocked groups (no shock-GFP-Veh and no shock-DRβ-Veh, p<.01, respectively), suggesting that exposure to shock and reminders upregulated CB1r protein expression. However, the Shock-GFP-URB group demonstrated a significant downregulation in CB1r compared to the no shock-GFP-URB group (p<.01). Also, the shock-DRβ-URB group demonstrated a significant downregulation in CB1r compared to the Shock-GFP-Veh group (p<.05).
In addition, non-stressed URB-treated rats (no shock-GFP-URB and no shock- DRβ-URB) demonstrated a significant upregulation in CB1r expression compared to non-treated groups (no shock-GFP-Veh: p<.001 and no shock-DRβ-Veh: p<.05, respectively), indicating that URB upregulated CB1r expression in non-stressed rats. Finally, the no shock-DRβ-Veh group demonstrated a significant upregulation in CB1r compared to the no shock-GFP-Veh group (p<.05), and the no shock-DRβ-URB group demonstrated significant downregulation in CB1r levels compared to the no shock-GFP-URB group (p<.001).
3.2.10. β-Catenin
3.2.10.1. PFC
β-catenin expression was measured in the mPFC (Figure 4i; n=7-9). A mixed design three- way ANOVA [shock×virus×drug; 2×2×2)] revealed significant effects of shock (F(1,56)=14.65, p<.001), virus (F(1,56)=11.31, p<.001), drug (F(1,56)=32.39, p<.001), shock×drug (F(1,56)=5.15, p<.05) and virus×drug (F(1,56)=23.79, p<.001) interactions. Post-hoc analysis revealed that the stressed groups (shock-GFP-Veh and shock-DRβ-Veh) demonstrated a significant downregulation in β-catenin protein levels compared to the non-shocked groups (no shock-GFP-Veh: p<.001 and no shock-DRβ-Veh: p<.05, respectively), suggesting that exposure to shock and reminders downregulated β-catenin protein expression in the mPFC.
However, the shock-GFP-URB group demonstrated a significant upregulation in β-catenin compared to the shock-GFP-Veh and shock-DRβ-URB (p<.001) groups, suggesting that URB normalized the shock- and reminders-induced downregulation in β-catenin, and that downregulating β-catenin in the mPFC blocked the therapeutic-like effect of URB. In addition, the no shock-GFP-URB group demonstrated a significant upregulation in β-catenin protein levels compared to the no shock-GFP-Veh and no shock-DRβ-URB groups (p<.01).
3.2.10.2. BLA
β-catenin expression was measured in the BLA (Figure 4j; n=8-9). A mixed design three- way ANOVA [shock×virus×drug; 2×2×2] revealed significant effects of shock (F(1,56)=12.98, p<.001), drug (F(1,56)=7.55, p<.01), shock×drug (F(1,56)=27.93, p<.001), virus×drug (F(1,56)=99.05, p<.001), shock×virus (F(1,56)=13.84, p<.001) and shock×virus×drug (F(1,56)=7.83, p<.01) interactions. Post-hoc analysis revealed that the shock-GFP-Veh group demonstrated a significant upregulation in β-catenin protein levels compared to the no shock-GFP-Veh group (p<.001).
However, the shock-GFP-URB group demonstrated a significant downregulation in β-catenin compared to the shock-GFP-Veh and shock-DRβ-URB groups (p<.001), suggesting that URB normalized the shock- and reminders-induced upregulation in β-catenin, and that downregulating β-catenin in the mPFC blocked the therapeutic-like effect of URB. Also, the shock-DRβ-Veh group demonstrated a significant downregulation in β-catenin expression compared to the shock-GFP-Veh group (p<.001). In addition, the no shock-DRβ-URB group demonstrated a significant upregulation in β-catenin protein levels compared to the no shock-GFP-URB and no shock-DRβ-Veh groups (p<.001); and the no shock-GFP-Veh group demonstrated a significant upregulation in β-catenin compared to the no shock-GFP-URB (p<.01) and no shock-DRβ-Veh (p<.05) groups.
4. Discussion
Our findings show that the restoring effects of URB597 on mPFC-CRF and CB1r are mediated through β-catenin activation in the mPFC, suggesting a new mechanism that mediates the stress-protective effects of URB597 in a PTSD model. Viral downregulation of mPFC-β-catenin in rats exposed to shock and reminders also blocked the restoring effects of URB597 on the development of an anxiogenic behavioral phenotype.
4.1. The Effects of URB597 on the Stress Markers
Exposure to the shock and reminders model of PTSD upregulated the expression of stress markers (CRF, CRFr1, and GR) and CB1r in the brain’s fear circuit, and induced anxiety-like behavior, deficits in social behavior, and impaired extinction learning. The upregulation of CRF, CRFr1, and GR in the mPFC and BLA was positively correlated with freezing behavior during exposure to the first SR. URB597 administered one hr after shock exposure prevented the shock- and reminders-induced alterations in behavior (anxiety, social interaction, and freezing) and the effects of the shock on the stress markers in the mPFC and BLA, as well as the upregulation in CB1r. These results are consistent with our previous findings demonstrating a therpeutic-like effect of URB597 that alleviated the actions of shock and reminders on anxiety- and depression-like behaviors, plasticity in the BLA and hippocampus, alterations in brain-derived neurotrophic factor (BDNF) and CB1r [25, 29, 53].
The upregulation in CRF and CRFr1 levels in the mPFC and BLA after exposure to shock and reminders, which was associated with anxiety-like behavior, corroborates other studies [3, 5, 18, 25, 27, 31, 32, 39, 58]. In humans, PTSD patients show upregulated CRF expression in the cerebrospinal fluid (CSF), attributable to CRF overexpression from extrahypothalamic sources such as the central amygdala [60-64]. PTSD patients show brain CRF hypersecretion and HPA-axis dysregulation while presenting startle hyperreactivity in response to stressful situations [65]. Preclinical studies showed that acute and chronic stress increased BLA-CRF-binding protein gene expression [66], and was correlated with sustained elevation in CRF1r expression [67]. Exposure to inescapable electric foot shock as a PTSD model or a single prolonged stress resulted in increased CRFr1 protein expression in the PFC and amygdala [25, 32]. Moreover, predator odor stress upregulated mPFC CRF cell counts of rats that avoided a stress-paired context, and CRF infusions in the vmPFC resulted in conditioned avoidance [3]. Moreover, CRFr1 antagonist administration in the mPFC blocked CRF signaling reversing stress-paired avoidance [3] and mitigating hyper-arousal symptoms in stressed rats [68]. Another study showed fear extinction memory is impaired by the upregulation of endogenous CRF in the amygdala as well as by intra-BLA CRF infusions [69]. Moreover, intra-BLA infusions of CRF resulted in a behavioral profile identical to that seen with predator exposure resembling aspects of PTSD as an exaggerated startle [70]. Altogether, these findings suggest that increased CRF signaling may regulate PTSD-related phenotypes.
It has been suggested that CRF and endocannabinoids regulate behavioral and hormonal stress responses in an opposing manner, hence ECB activation terminates the stress response and CRF activation promotes an anxiogenic behavioral phenotype [71]. Chronic stress upregulates CRF in the amygdala and PFC, which in turn increases FAAH activity that results in an AEA deficient state and an anxiogenic phenotype, suggesting that CRF regulates stress-induced alterations in ECB signaling [4, 28]. Moreover, a CB1r-dependent mechanism in the mPFC was suggested to mediate the termination of HPA axis activation following exposure to stress [72]. CB1r mRNA was found co-localized with CRF mRNA both in the hypothalamic nuclei of the paraventricular nucleus [73] and in other extrahypothalamic areas in the brain`s fear circuit, such as PFC and amygdala [74]. Pharmacological and knockout studies show that CB1r activity limits hypothalamic CRF release [73]. We found that activation of mPFC-CB1r with FAAH inhibition downregulated the expression of mPFC-CRF and terminated the stress response, supporting that enhancing ECB signaling modulates cortical CRF following stress.
4.2. The Effects of Downregulating β-catenin on URB597 Modulation of the Stress Markers and CB1
Viral-mediated mPFC downregulation of β-catenin function blocked the therapeutic-like effect of URB597 on behavior, corroborating previous studies from our lab that targeted the NAc [30, 75]. We previousely showed that inhibition of β-catenin in the NAc using the non-selective β-catenin antagonist sulindac or downregulating β-catenin activity using a viral approach blocked the therapeutic effects of enhancing ECB signaling on anxiognic- and depressive-like behavior [30, 75]. Importantly, here we show that downregulating mPFC-β-catenin blocks the ameliortaing effects of URB597 on the stress-induced increase in CRF and CB1r. We have previousely suggested therapeutic-like effects of URB597, acting through CB1r to modulate β-catenin and produce pro-resilient responses [30]. CB1rs, which are predominantly localized to gamma-aminobutyric acid (GABA)-positive interneurons, have a key role in regulating PFC activity and stress response termination [72, 76].
A strong functional interaction between CB1r and β-catenin has been suggested [30]. CB1r activation increases PI3K/AKT activity leading to GSK-3β phosphorylation, β-catenin stabilization, and its translocation into the nucleus; in the nucleus, β-catenin regulates transcription and gene expression to promote anti-stress responses [54, 35, 49, 54]. Hence, URB597, acting through CB1r, modulates β-catenin that produces pro-resilient responses.
β-catenin expression was decreased in the mPFC and increased in the BLA following shock and reminders, and this was restored by URB597. Only in the mPFC, the restoring effects of URB597 were blocked by β-catenin downregulation. It has been shown that resveratrol reduced CRF mRNA expression in the hypothalamus of stressed rats and upregulated the relative ratio of phosphorylated (p)-GSK3β/GSK3β and protein levels of p-GSK3β, cyclin D1, and c-myc, while downregulating the relative ratio of p-β-catenin/β-catenin and expression of GSK3β in the hippocampus. The authors suggested that the antidepressant-like effects observed for resveratrol were obtained by downregulation of the HPA axis hyperactivity and regulating the Wnt/β-catenin pathway [77]. Together with our findings, this suggests a functional relationship between β-catenin and CRF that needs to be further investigated.
5. Conclusion
In conclusion, our results demonstrate that URB597 prevents the development of an anxiogenic phenotype in rats exposed to shock and reminders due to regulation of CRF via CB1r and the Wnt/β-catenin pathway in the mPFC.
Supplementary Materials
The following supporting information can be downloaded at: www.mdpi.com/xxx/s1, Table S1: Primers for mRNAs used for real-time PCR; Figure S1: An illustration of modified HSV amplicon plasmid; Figure S1b: The effects of the FAAH inhibitor URB597 on gene expression of CRF, GR and CRFr1 in the mPFC and BLA in rats exposed to shock and reminders.
Author Contributions
Conceptualization: I.A. and B.S.; methodology: B.S., A.P and T.M.Z; formal analysis: B.S.; investigation: B.S.; resources: I.A. E.N.; writing-original draft preparation: B.S. and A.P.; writing-review and editing: A.P., I.A. E.P and E.N; visualization: B.S. A.P.; supervision: I.A.; funding acquisition: I.A. All authors have read and agreed to the published version of the manuscript.
Funding
This research was funded by the Israel Science Foundation (ISF), grant number 993/20 to IA.
The Institutional Review Board Statement
The animal study protocol was approved by University of Haifa Ethics and Animal Care Committee and appropriate measures were taken to minimize pain and discomfort [protocol codes 403 (2016), 535 (2017) and 600 (2018)].
Data Availability Statement
Data sharing is not applicable to this article.
Conflicts of Interest
Conflicts of Interest
REFERENCES
[1] Nurit Aisenberg, Lidia Serova, Esther
L Sabban, et al. “The effects of enhancing endocannabinoid signaling and
blocking corticotrophin releasing factor receptor in the amygdala and
hippocampus on the consolidation of a stressful event.” Eur Neuropsychopharmacol,
vol. 27, no. 9, pp. 913-927, 2017. View at: Publisher Site | PubMed
[2] Stephen C Heinrichs, George F Koob
“Corticotropin-releasing factor in brain: a role in activation, arousal, and
affect regulation.” J Pharmacol Exp Ther, vol. 311, no. 2, pp. 427-440,
2004. View at: Publisher
Site | PubMed
[3] Allyson L Schreiber, Yi-Ling Lu,
Brittni B Baynes, et al. “Corticotropin-releasing factor in ventromedial
prefrontal cortex mediates avoidance of a traumatic stress-paired context.” Neuropharmacology,
vol. 113, pp. 323-330, 2017. View at: Publisher Site | PubMed
[4] J Megan Gray, Christopher D Wilson,
Tiffany T Y Lee, et al. “Sustained glucocorticoid exposure recruits
cortico-limbic CRH signaling to modulate endocannabinoid function.” Psychoneuroendocrinology,
vol. 66, pp. 151-158, 2016. View at: Publisher Site | PubMed
[5] Irit Akirav “Cannabinoids and
glucocorticoids modulate emotional memory after stress.” Neurosci Biobehav Rev,
vol. 37, no. 10, pp. 2554-2563, 2013. View at: Publisher Site | PubMed
[6] Cecilia J Hillard “Stress regulates
endocannabinoid-CB1 receptor signaling.” Semin Immunol, vol. 26, no. 5,
pp. 380-388, 2014. View at: Publisher
Site | PubMed
[7] Veronika Kondev, Mustafa Najeed,
Farhana Yasmin, et al. “Endocannabinoid Release at Ventral Hippocampal-Amygdala
Synapses Regulates Stress-Induced Behavioral Adaptation.” SSRN, pp.
4339779, 2023. View at: Publisher
Site
[8] Beat Lutz, Giovanni Marsicano, Rafael
Maldonado, et al. “The endocannabinoid system in guarding against fear, anxiety
and stress.” Nat Rev Neurosci, vol. 16, no. 12, pp. 705-718, 2015. View
at: Publisher Site | PubMed
[9] Fabrício A Moreira, Carsten T Wotjak
“Cannabinoids and anxiety.” Curr Top Behav Neurosci, vol. 2, pp.
429-450, 2010. View at: Publisher
Site | PubMed
[10] Sachin Patel, Mathew N Hill, Joseph F
Cheer, et al. “The endocannabinoid system as a target for novel anxiolytic
drugs.” Neurosci Biobehav Rev, vol. 76, pp. 56-66, 2017. View at: Publisher Site | PubMed
[11] Jennifer Spohrs, Michael Prost,
Martin Ulrich, et al. “Endocannabinoid system reactivity during stress
processing in healthy humans.” Biol Psychol, vol. 169, pp. 108281, 2022.
View at: Publisher
Site | PubMed
[12] Andrea Berardi, Gustav Schelling,
Patrizia Campolongo “The endocannabinoid system and Post Traumatic Stress
Disorder (PTSD): From preclinical findings to innovative therapeutic approaches
in clinical settings.” Pharmacol Res, vol. 111, pp. 668-678., 2016 View
at: Publisher
Site | PubMed
[13] Matthew N Hill, Patrizia Campolongo,
Rachel Yehuda, et al. “Integrating endocannabinoid signaling and cannabinoids
into the biology and treatment of posttraumatic stress disorder.” Neuropsychopharmacology,
vol. 43, no. 1, pp. 80-102, 2018. View at: Publisher Site | PubMed
[14] Nachshon Korem, Tomer Mizrachi
Zer-Aviv, Eti Ganon-Elazar, et al. Targeting the endocannabinoid system to
treat anxiety-related disorders. J Basic Clin Physiol Pharmacol, vol.
27, no. 3, pp. 193-202, 2016. View at: Publisher Site | PubMed
[15] Sabrina F Lisboa, C Vila-Verde, J
Rosa, et al. “Tempering aversive/traumatic memories with cannabinoids: a review
of evidence from animal and human studies.” Psychopharmacology, vol.
236, no. 1, pp. 201-226, 2019. View at: Publisher Site | PubMed
[16] Mallory Je Loflin, Kimberly A Babson,
Marcel O Bonn-Miller “Cannabinoids as therapeutic for PTSD.” Curr Opin
Psychol, vol 14, pp. 78-83, 2017. View at: Publisher Site | PubMed
[17] Leah M Mayo, Christine A Rabinak,
Matthew N Hill, et al. “Targeting the endocannabinoid system in the treatment
of posttraumatic stress disorder: A promising case of preclinical-clinical
translation?” Biol Psychiatry, vol. 91, no. 3, pp. 262-272, 2022. View
at: Publisher
Site | PubMed
[18] Brenda Sbarski, Irit Akirav
“Cannabinoids as therapeutics for PTSD.” Pharmacol Ther, vol. 211, pp.
107551, 2020. View at: Publisher Site | PubMed
[19] Maria M Steenkamp, Esther M Blessing,
Isaac R Galatzer-Levy, et al. “Marijuana and other cannabinoids as a treatment
for posttraumatic stress disorder: A literature review.” Depress Anxiety,
vol. 34, no. 3, pp. 207-216, 2017. View at: Publisher Site | PubMed
[20] Georgia Balsevich, Gavin N Petrie,
Matthew N Hill, et al. “Endocannabinoids: Effectors of glucocorticoid
signaling.” Front Neuroendocrinol, vol. 47, pp. 86-108, 2017. View at: Publisher Site | PubMed
[21] Cecilia J Hillard, Margaret Beatka,
Jenna Sarvaideo “Endocannabinoid signaling and the
hypothalamic-pituitary-adrenal axis.” Compr Physiol, vol. 7, no. 1, pp.
1-15, 2016. View at: Publisher
Site | PubMed
[22] Maria Morena, Sachin Patel, Jaideep S
Bains, et al. “Neurobiological interactions between stress and the
endocannabinoid system.” Neuropsychopharmacology, vol. 41, no. 1, pp.
80-102, 2016. View at: Publisher
Site | PubMed
[23] Eti Ganon-Elazar, Irit Akirav
“Cannabinoids prevent the development of behavioral and endocrine alterations
in a rat model of intense stress.” Neuropsychopharmacology, vol 37, no.
2, pp. 456-466, 2012. View at: Publisher
Site | PubMed
[24] Sachin Patel, Craig T Roelke, David J
Rademacher, et al. “Endocannabinoid signaling negatively modulates
stress-induced activation of the hypothalamic-pituitary-adrenal axis.” Endocrinology,
vol. 145, no. 12, pp. 5431-5438, 2004. View at: Publisher Site | PubMed
[25] Noa Shoshan, Irit Akirav “The effects
of cannabinoid receptors activation and glucocorticoid receptors deactivation
in the amygdala and hippocampus on the consolidation of a traumatic event.” Neurobiol
Learn Mem, vol. 144, pp. 248-258, 2017. View at: Publisher Site | PubMed
[26] Chris S Breivogel, Laura J Sim-Selley
“Basic neuroanatomy and neuropharmacology of cannabinoids.” Int Rev
Psychiatry, vol. 21, no. 2, pp. 113-121, 2009. View at: Publisher Site | PubMed
[27] María Ruth Pazos, Estefanía Núñez,
Cristina Benito, et al. “Functional neuroanatomy of the endocannabinoid
system.” Pharmacol Biochem Behav, vol. 81, no. 2, pp. 239-247, 2005.
View at: Publisher
Site | PubMed
[28] A L Gray, T M Hyde, A Deep-Soboslay,
et al. “Sex differences in glutamate receptor gene expression in major
depression and suicide.” Mol Psychiatry, vol. 20, no. 9, pp. 1057-1068,
2015. View at: Publisher
Site | PubMed
[29] Or Burstein, Noa Shoshan, Ravid
Doron, et al. “Cannabinoids prevent depressive-like symptoms and alterations in
BDNF expression in a rat model of PTSD.” Prog Neuropsychopharmacol Biol
Psychiatry, vol. 84, pp. 129-139, 2018. View at: Publisher Site | PubMed
[30] Tomer Mizrachi Zer-Aviv, Larglinda
Islami, Peter J Hamilton, et al. “Enhancing Endocannabinoid Signaling via
β-Catenin in the Nucleus Accumbens Attenuates PTSD- and
Depression-like Behavior of Male Rats.” Biomedicines, vol. 10, no. 8,
pp. 1789, 2022. View at: Publisher
Site | PubMed
[31] Amir Segev, Nachshon Korem, Tomer
Mizrachi Zer-Aviv, et al. “Role of endocannabinoids in the hippocampus and
amygdala in emotional memory and plasticity.” Neuropsychopharmacology,
vol. 43, no. 10, pp. 2017-2027, 2018. View at: Publisher Site | PubMed
[32] Noa Shoshan, Amir Segev, Hila Abush,
et al. “Cannabinoids prevent the differential long‐term effects of exposure to severe stress on hippocampal‐and amygdala‐dependent memory and plasticity.” Hippocampus,
vol. 27, no. 10, pp. 1093-1109, 2017. View at: Publisher Site | PubMed
[33] Chuin Hau Teo, Tomoko Soga, Ishwar S
Parhar “Brain beta-catenin signalling during stress and depression.” Neurosignals,
vol. 26, no. 1, pp. 31-42, 2018. View at: Publisher Site | PubMed
[34] Alexandre Vallée “Neuroinflammation
in schizophrenia: the key role of the WNT/β-catenin pathway.” Int J Mol Sci, vol. 23, no.
5, pp. 2810, 2022. View at: Publisher
Site | PubMed
[35] Ning Xu, Lian Geng 2, Xianxia Yan
“Involvement of canonical Wnt/β-catenin signaling in the extinction of auditory fear
conditioning in male mice.” Behav Brain Res, vol. 445, pp. 114378, 2023. View at:
Publisher Site | PubMed
[36] Kimberly A Maguschak, Kerry J Ressler
“A role for WNT/β-catenin signaling in the neural mechanisms of behavior.” J
Neuroimmune Pharmacol, vol. 7, no. 4, pp. 763-773, 2012. View at: Publisher Site | PubMed
[37] Rodrigo F Narvaes, Eduarda G
Nachtigall, Lucas A Marcondes, et al. “Involvement of medial prefrontal cortex
canonical Wnt/β-catenin and non-canonical Wnt/Ca2+ signaling pathways in contextual fear
memory in male rats.” Behav Brain Res, vol. 430, pp. 113948, 2022. View at: Publisher Site | PubMed
[38] Félicien Karege, Nader Perroud, Sandra
Burkhardt, et al. “Protein levels of β-catenin and activation state of
glycogen synthase kinase-3β in major depression. A study with postmortem
prefrontal cortex.” J Affect Disord, vol. 136, no. 1-2, pp. 185-188, 2012. View at: Publisher Site | PubMed
[39] Fuencisla Pilar-Cúellar, Rebeca
Vidal, Alvaro Díaz, et al. “Signaling pathways involved in
antidepressant-induced cell proliferation and synaptic plasticity.” Curr
Pharm Des, vol. 20, no. 23, pp. 3776-3794, 2014. View at: Publisher Site | PubMed
[40] Xinguo Ren, Hooriyah S Rizavi,
Mansoor A Khan, et al. “Altered Wnt signalling in the teenage suicide brain:
focus on glycogen synthase kinase-3β and β-catenin.” Int J Neuropsychopharmacol,
vol. 16, no. 5, pp. 945-955, 2013. View at: Publisher Site | PubMed
[41] Y C Chen, Q R Tan, W Dang, et al.
“The effect of citalopram on chronic stress-induced depressive-like behavior in
rats through GSK3β/β-catenin activation in the medial prefrontal cortex.” Brain Res Bull, vol. 88, no.
4, pp. 338-344, 2012. View at: Publisher
Site | PubMed
[42] Santiago Cuesta, Alejandrina Funes,
Alejandra M Pacchioni “Social isolation in male rats during adolescence
inhibits the Wnt/β-catenin pathway in the prefrontal cortex and enhances anxiety and
cocaine-induced plasticity in adulthood.” Neurosci Bull, vol. 36, no. 6, pp. 611-624,
2020. View at: Publisher
Site | PubMed
[43] Mohamed Z Habib, Mai A Ebeid, Yasser
El Faramawy, et al. “Effects of lithium on cytokine neuro-inflammatory
mediators, Wnt/β-catenin signaling and microglial activation in the hippocampus of
chronic mild stress-exposed rats.” Toxicol Appl Pharmacol, vol. 399, pp. 115073,
2020. View at: Publisher
Site | PubMed
[44] Yea-Hyun Leem, Morimasa Kato, Hyukki
Chang “Regular exercise and creatine supplementation prevent chronic mild
stress-induced decrease in hippocampal neurogenesis via Wnt/GSK3β/β-catenin pathway.” J Exerc Nutrition Biochem,
vol. 22, no. 2, pp. 1-6, 2018. View at: Publisher Site | PubMed
[45] Ahmed M Mohamed, Mohamed Z Habib 1,
Mai A Ebeid, et al. “Amisulpride alleviates chronic mild stress-induced
cognitive deficits: Role of prefrontal cortex microglia and Wnt/β-catenin pathway.” Eur J Pharmacol, vol. 885,
pp. 173411, 2020. View at: Publisher Site | PubMed
[46] Caroline Dias, Jian Feng, Haosheng
Sun, et al. “β-catenin mediates stress resilience through Dicer1/microRNA regulation.” Nature,
vol. 516, no. 7529, pp. 51-55, 2014. View at: Publisher Site | PubMed
[47] Oksana Kaidanovich-Beilin, Anat
Milman, Abraham Weizman, et al. “Rapid antidepressive-like activity of specific
glycogen synthase kinase-3 inhibitor and its effect on β-catenin in mouse hippocampus.” Biol
Psychiatry, vol. 55, no. 8, pp. 781-784, 2004. View at: Publisher Site | PubMed
[48] Rebeca Vidal, Emilio Garro-Martínez,
Álvaro Díaz, et al. “Targeting β-catenin in GLAST-expressing cells: impact on anxiety
and depression-related behavior and hippocampal proliferation.” Mol Neurobiol, vol. 56, no.
1, pp/ 553-566, 2019. View at: Publisher Site | PubMed
[49] Matthew B Wilkinson, Caroline Dias,
Jane Magida, et al. “A novel role of the WNT-dishevelled-GSK3β signaling cascade in the mouse
nucleus accumbens in a social defeat model of depression.” J Neurosci, vol. 31, no. 25,
pp. 9084-9092, 2011. View at: Publisher Site | PubMed
[50] M Dahlhoff, A Siegmund, Y Golub, et
al. “AKT/GSK-3β/β-catenin signalling within hippocampus and amygdala reflects genetically
determined differences in posttraumatic stress disorder like symptoms.” Neuroscience, vol. 169, no.
3, pp. 1216-1226, 2010. View at: Publisher
Site | PubMed
[51] Irit Akirav, Amir Segev, Helen
Motanis, et al. “D-cycloserine into the BLA reverses the impairing effects of
exposure to stress on the extinction of contextual fear, but not conditioned
taste aversion.” Learn Mem, vol. 16, no. 11, pp. 682-686, 2009. View at:
Publisher Site | PubMed
[52] M S Fanselow “Conditional and
unconditional components of post-shock freezing.” Pavlov J Biol Sci,
vol. 15, no. 4, pp. 177-182, 1980. View at: Publisher Site | PubMed
[53] Shirley Alteba, Tomer Mizrachi
Zer-Aviv, Adi Tenenhaus, et al. “Antidepressant-like effects of URB597 and
JZL184 in male and female rats exposed to early life stress.” Eur
Neuropsychopharmacol, vol. 39, pp. 70-86, 2020. View at: Publisher Site | PubMed
[54] Neta Maymon, Tomer Mizrachi Zer-Aviv,
Esther L Sabban, et al. “Neuropeptide Y and cannabinoids interaction in the
amygdala after exposure to shock and reminders model of PTSD.” Neuropharmacology,
vol. 162, pp. 107804, 2020. View at: Publisher Site | PubMed
[55] Nachshon Korem, Irit Akirav
“Cannabinoids prevent the effects of a footshock followed by situational
reminders on emotional processing.” Neuropsychopharmacology, vol. 39,
no. 12, pp. 2709-2722, 2014. View at: Publisher Site | PubMed
[56] Hagar Bauminger, Hiba Zaidan, Irit
Akirav “Anandamide Hydrolysis Inhibition Reverses the Long-Term Behavioral and
Gene Expression Alterations Induced by MK-801 in Male Rats: Differential CB1
and CB2 Receptor-Mediated Effects.” Schizophr Bull, vol. 48, no. 4, pp.
795-803, 2022. View at: Publisher
Site | PubMed
[57] Hiba Zaidan, Gokul Ramaswami, Yaela N
Golumbic, et al. “A-to-I RNA editing in the rat brain is age-dependent,
region-specific and sensitive to environmental stress across generations.” BMC
Genomics, vol. 19, no. 1, pp. 28, 2018. View at: Publisher Site | PubMed
[58] Michael W Pfaffl, Ales Tichopad,
Christian Prgomet, et al. “Determination of stable housekeeping genes,
differentially regulated target genes and sample integrity:
BestKeeper-Excel-based tool using pair-wise correlations.” Biotechnol Lett,
vol. 26, no. 6, pp. 509-515, 2004. View at: Publisher
Site | PubMed
[59] Gregory J Gage, Daryl R Kipke,
William Shain “Whole animal perfusion fixation for rodents.” J Vis Exp,
no. 65, pp. 3564, 2012. View at: Publisher
Site | PubMed
[60] Dewleen G Baker, Nosa N Ekhator, John
W Kasckow, et al. “Higher levels of basal serial CSF cortisol in combat
veterans with posttraumatic stress disorder.” Am J Psychiatry, vol. 162,
no. 5, pp. 992-994, 2005. View at: Publisher Site | PubMed
[61] E B Binder, C B Nemeroff “The CRF
system, stress, depression and anxiety—insights from human genetic studies.” Mol
Psychiatry, vol. 15, no. 6, pp. 574-588, 2010. View at: Publisher Site | PubMed
[62] J D Bremner, J Licinio, A Darnell, et
al. “Elevated CSF corticotropin-releasing factor concentrations in
posttraumatic stress disorder.” Am J Psychiatry, vol. 154, no. 5, pp.
624-629, 1997. View at: Publisher
Site | PubMed
[63] C S de Kloet, E Vermetten, E Geuze,
et al. “Elevated plasma corticotrophin-releasing hormone levels in veterans
with posttraumatic stress disorder.” Prog Brain Res, vol. 167, pp.
287-291, 2008. View at: Publisher
Site | PubMed
[64] Frederic J Sautter, Garth Bissette,
Justin Wiley, et al. “Corticotropin-releasing factor in posttraumatic stress
disorder (PTSD) with secondary psychotic symptoms, nonpsychotic PTSD, and
healthy control subjects.” Biol Psychiatry, vol. 54, no. 12, pp.
1382-1388, 2003. View at: Publisher Site | PubMed
[65] Victoria B Risbrough, Murray B Stein
“Role of corticotropin releasing factor in anxiety disorders: a translational
research perspective.” Horm Behav, vol. 50, no. 4, pp. 550-561, 2006.
View at: Publisher
Site | PubMed
[66] Ryan J Herringa, Steven A Nanda,
David T Hsu, et al. “The effects of acute stress on the regulation of central
and basolateral amygdala CRF-binding protein gene expression.” Brain Res Mol
Brain Res, vol. 131, no. 1-2, pp. 17-25, 2004. View at: Publisher
Site | PubMed
[67] Einat Elharrar, Gal Warhaftig, Orna
Issler, et al. “Overexpression of corticotropin-releasing factor receptor type
2 in the bed nucleus of stria terminalis improves posttraumatic stress
disorder-like symptoms in a model of incubation of fear.” Biol Psychiatry,
vol. 74, no. 11, pp. 827-836, 2013. View at: Publisher Site | PubMed
[68] Emily A Roltsch, Brittni B Baynes,
Jacques P Mayeux, et al. “Predator odor stress alters corticotropin-releasing
factor-1 receptor (CRF1R)-dependent behaviors in rats.” Neuropharmacology,
vol. 79, pp. 83-89, 2014. View at: Publisher Site | PubMed
[69] Dina Abiri, Christina E Douglas,
Katina C Calakos, et al. “Fear extinction learning can be impaired or enhanced
by modulation of the CRF system in the basolateral nucleus of the amygdala.” Behav
Brain Res, vol. 271, pp. 234-239, 2014. View at: Publisher Site | PubMed
[70] Abha K Rajbhandari, Brian A Baldo,
Vaishali P Bakshi “Predator stress-induced CRF release causes enduring
sensitization of basolateral amygdala norepinephrine systems that promote
PTSD-like startle abnormalities.” J Neurosci, vol. 35, no. 42, pp.
14270-14285, 2015. View at: Publisher Site | PubMed
[71] Julia Ruat, Alice Hartmann 3, Daniel
E Heinz, et al. “CB1 receptors in corticotropin‐releasing
factor neurons selectively control the acoustic startle response in male mice.”
Genes Brain Behav, vol. 20. No. 8, pp. e12775, 2021. View at: Publisher Site | PubMed
[72] Matthew N Hill, Ryan J McLaughlin,
Bin Pan, et al. “Recruitment of prefrontal cortical endocannabinoid signaling
by glucocorticoids contributes to termination of the stress response.” J
Neurosci, vol. 31, no. 29, pp. 10506-10515, 2011. View at: Publisher Site | PubMed
[73] Daniela Cota, Giovanni Marsicano,
Matthias Tschöp, et al. “The endogenous cannabinoid system affects energy
balance via central orexigenic drive and peripheral lipogenesis.” J Clin
Invest, vol. 112, no. 3, pp. 423-431, 2003. View at: Publisher Site | PubMed
[74] Daniela Cota, Michel-Alexander
Steiner, Giovanni Marsicano, et al. “Requirement of cannabinoid receptor type 1
for the basal modulation of hypothalamic-pituitary-adrenal axis function.” Endocrinology,
vol. 148, no. 4, pp. 1574-1581, 2007. View at: Publisher Site | PubMed
[75] Nachshon Korem, Rachel Lange, Cecilia
J Hillard, et al. “Role of beta-catenin and endocannabinoids in the nucleus
accumbens in extinction in rats exposed to shock and reminders.” Neuroscience,
vol. 357, pp. 285-294, 2017 View at: Publisher
Site | PubMed
[76] Ryan J McLaughlin, Matthew N Hill,
Boris B Gorzalka “A critical role for prefrontocortical endocannabinoid
signaling in the regulation of stress and emotional behavior.” Neurosci
Biobehav Rev, vol. 42, pp. 116-131, 2014. View at: Publisher Site | PubMed
[77] Jin-Fang Ge, Ya-Yun Xu, Gan Qin, et
al. “Resveratrol ameliorates the anxiety-and depression-like behavior of
subclinical hypothyroidism rat: possible involvement of the HPT axis, HPA axis,
and Wnt/β-catenin pathway.” Front Endocrinol (Lausanne), vol. 7, pp. 44, 2016. View at: Publisher Site | PubMed
[78] Paxinos G., Watson C. “The Rat Brain
in Stereotaxic Coordinates Fourth Edition.” Academic press, 1998.
[79] Lin Chen, Song Li, Jie Cai, et al.
“Activation of CRF/CRFR1 signaling in the basolateral nucleus of the amygdala
contributes to chronic forced swim-induced depressive-like behaviors in rats.” Behav
Brain Res, vol. 338, pp. 134-142, 2018. View at: Publisher Site | PubMed
[80] Eti Ganon-Elazar, Irit Akirav
“Cannabinoids and traumatic stress modulation of contextual fear extinction and
GR expression in the amygdala-hippocampal-prefrontal circuit.” Psychoneuroendocrinology,
vol. 38, no. 9, pp. 1675-1687, 2013. View at: Publisher Site | PubMed
[81] Chuting Li, Yuan Liu, Shiping Yin, et
al. “Long-term effects of early adolescent stress: dysregulation of
hypothalamic-pituitary-adrenal axis and central corticotropin releasing factor
receptor 1 expression in adult male rats.” Behav Brain Res, vol. 288,
pp. 39-49, 2015. View at: Publisher
Site | PubMed
[82] Anna Skórzewska, Małgorzata Lehner,
Aleksandra Wisłowska-Stanek, et al. “Individual susceptibility or resistance to
posttraumatic stress disorder-like behaviours.” Behav Brain Res, vol.
386, pp. 112591, 2020. View at: Publisher Site | PubMed
[83] Stefania Trazzi, Martin Steger,
Valentina Maria Mitrugno, et al. “CB1 cannabinoid receptors increase neuronal
precursor proliferation through AKT/glycogen synthase kinase-3β/β-catenin signaling.” J Biol Chem, vol. 285, no.
13, pp. 10098-10109, 2010. View at: Publisher Site | PubMed
[84] Rebeca Vidal, Fuencisla
Pilar-Cuéllar, Severiano dos Anjos, et al. “New strategies in the development
of antidepressants: towards the modulation of neuroplasticity pathways.” Curr
Pharm Des, vol. 17, no. 5, pp. 521-533, 2011. View at: Publisher Site | PubMed
[85] Sheng-Chiang Wang, Chen-Cheng Lin, Nian-Sheng Tzeng, et al. “Effects of oxytocin on prosocial behavior and the associated profiles of oxytocinergic and corticotropin-releasing hormone receptors in a rodent model of posttraumatic stress disorder.” J Biomed Sci, vol. 26, no. 1, pp. 1-12, 2019. View at: Publisher Site | PubMed
[86] Marcus M Weera, Allyson L Schreiber, Elizabeth M Avegno “The role of central amygdala corticotropin-releasing factor in predator odor stress-induced avoidance behavior and escalated alcohol drinking in rats.” Neuropharmacology, vol. 166, pp. 107979, 2020. View at: Publisher Site | PubMed